Hope For CO2 Removal
This study explores how countries can achieve net-zero targets by addressing hard-to-abate CO2 emissions through carbon dioxide removal (CDR). The assessment focuses on 14 CDR options in Germany, evaluating their feasibility based on technological, economic, environmental, social-cultural, and institutional aspects. It highlights challenges and opportunities for implementing CDR strategies towards climate goals.
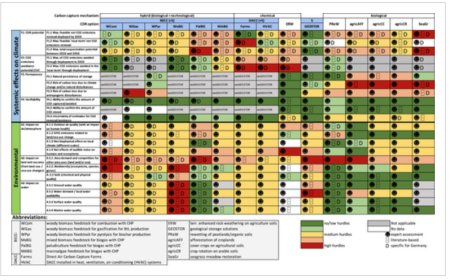
Countries aiming to achieve net-zero emissions will have to remove the remaining carbon dioxide from the atmosphere through carbon dioxide removal (CDR). However, current assessments of CDR options rarely consider socio-cultural or institutional aspects or set the CDR options in the specific context of their implementation. In this study, researchers conducted the first context-specific feasibility assessment of CDR options in Germany, considering six dimensions, including technological, economic, environmental, institutional, and social-cultural aspects. The study assessed 14 CDR options, including chemical carbon capture options, bioenergy combined with carbon capture and storage, and options to increase ecosystem carbon uptake. The study found that CDR options like cover crops or seagrass restoration face low implementation hurdles but have small CO2 removal potentials, while options like woody-biomass combustion or mixed-feedstock biogas production have high CDR potentials but face large economic and institutional hurdles. The analysis aims to provide comprehensive information on CDR options for use in further research and as an effective decision support basis for a range of actors. While Germany has been one of the most forward-thinking countries on the topic, they have to significantly increase their efforts to achieve their goals on Carbon emissions by 2045. Options to do so include peatland rewetting, afforestation and seagrass restoration.
Abstract
To reach their net-zero targets, countries will have to compensate hard-to-abate CO2 emissions through carbon dioxide removal (CDR). Yet, current assessments rarely include socio-cultural or institutional aspects or fail to contextualize CDR options for implementation. Here we present a context-specific feasibility assessment of CDR options for the example of Germany. We assess 14 CDR options, including three chemical carbon capture options, six options for bioenergy combined with carbon capture and storage (BECCS), and five options that aim to increase ecosystem carbon uptake. The assessment addresses technological, economic, environmental, institutional, social-cultural and systemic considerations using a traffic-light system to evaluate implementation opportunities and hurdles. We find that in Germany CDR options like cover crops or seagrass restoration currently face comparably low implementation hurdles in terms of technological, economic, or environmental feasibility and low institutional or social opposition but show comparably small CO2 removal potentials. In contrast, some BECCS options that show high CDR potentials face significant techno-economic, societal and institutional hurdles when it comes to the geological storage of CO2. While a combination of CDR options is likely required to meet the net-zero target in Germany, the current climate protection law includes a limited set of options. Our analysis aims to provide comprehensive information on CDR hurdles and possibilities for Germany for use in further research on CDR options, climate, and energy scenario development, as well as an effective decision support basis for various actors.
Key Points
-
More context-specific assessments of carbon dioxide removal (CDR) options are needed to guide national net-zero decision making
-
Ecosystem-based CDR options with comparably low implementation hurdles in Germany show relatively small CO2 removal potentials
-
High CDR potential options in Germany face high institutional, technological and societal hurdles linked in many ways to geological storage
Plain Language Summary
Countries aiming to achieve net-zero emissions will have to remove the remaining carbon dioxide from the atmosphere through carbon dioxide removal (CDR). However, current assessments of CDR options rarely consider socio-cultural or institutional aspects or set the CDR options in the specific context of their implementation. In this study, researchers conducted the first context-specific feasibility assessment of CDR options in Germany, considering six dimensions, including technological, economic, environmental, institutional, and social-cultural aspects. The study assessed 14 CDR options, including chemical carbon capture options, bioenergy combined with carbon capture and storage, and options to increase ecosystem carbon uptake. The study found that CDR options like cover crops or seagrass restoration face low implementation hurdles but have small CO2 removal potentials, while options like woody-biomass combustion or mixed-feedstock biogas production have high CDR potentials but face large economic and institutional hurdles. The analysis aims to provide comprehensive information on CDR options for use in further research and as an effective decision support basis for a range of actors.
1 Introduction
For Germany to reach its national climate targets of achieving net zero emissions by 2045 significant emission reductions are required (KSG, 2021). According to Mengis et al. (2021) the carbon budget Germany is allowed to emit to not exceed the goal of the Paris Agreement of limiting global warming to 1.5°C, equals 6.25 Gt from 1 January 2022 until net-zero. However, avoided (∼645 Mt CO2/year) and reduced (∼50 Mt CO2/year) emissions alone will not be sufficient for achieving those targets and approximately 60 Mt CO2 per year will need to be removed from the atmosphere through so-called carbon dioxide removal (CDR) methods (Mengis et al., 2022). CDR options—classified by the capturing process—include biological, chemical, and hybrid options, which either aim to enhance ecosystem productivity and related carbon sinks, chemical uptake mechanisms combined with carbon capture and storage (CCS), or point-source carbon capture from bioenergy plants (Borchers et al., 2022; see Section 2 for details). For CDR options to make a contribution to the national net zero target in Germany, significant upscaling of CDR options would be required (Mengis et al., 2022). Currently, Germany mentions three CDR options in their climate law: peatland rewetting, afforestation and seagrass restoration (KSG, 2021). The estimated scale of carbon removals from land-use, land-use change and forestry options in Germany amounts to 3 to 41 Mt CO2 per year by 2045 (see e.g., dena, 2021; Kopernikus-Projekt Ariadne, 2021). The question of scale is a complex issue that can be considered on many levels, including, but not limited to natural resources availability, land-use patterns, technical maturity, or storage potentials (Borchers et al., 2022; Fridahl et al., 2020). Thus, understanding the feasibility of reaching a particular scale of CDR options within their national context is crucial (Thoni et al., 2020).
The feasibility of deploying CDR options varies widely, for example, they come at different technology readiness levels (TRLs), are characterized by different CO2 removal potentials, and efficiencies, demand different types and amounts of resources, require variable investments, and generate different costs. They also impact the environment in different ways, and their public perception and legal framework for their deployment also vary. Selected aspects have been addressed in earlier CDR assessments (e.g., Dooley et al., 2020; Dow et al., 2015; Forster et al., 2020; Fuss et al., 2018; Honegger et al., 2021). When aiming for an extensive evaluation of CDR options, different aspects, for example, environmental, techno-economic, social, and institutional should be considered in conjunction. For this reason, we use a comprehensive assessment framework developed by Förster et al. (2022), which allows us to assess the feasibility of selected CDR options (Borchers et al., 2022) by identifying potential hurdles involved in CDR deployment (“effort for implementation”) and thereby also identifying potential “low-hanging-fruits” for possibly short-term implementation.
2 Methods
This assessment addresses the feasibility of CDR options for generating negative carbon emissions with the objective of achieving net-zero emissions in Germany. It includes CDR concepts that have been identified to be of relevance for achieving net-zero emissions in Germany by 2050 (Mengis et al., 2022) and are described in detail by Borchers et al. (2022). This assessment follows the framework developed by Förster et al. (2022) for assessing the feasibility of CDR options. The framework provides a comprehensive set of criteria and indicators together with a traffic light system for assessing the feasibility of CDR options related to environmental impacts and dependencies, their technological and economic requirements and consequences, social and institutional implications and the systemic contribution of CDR to climate change mitigation. Given the comprehensiveness of the addressed criteria and the diverse knowledge required for assessing the feasibility of CDR options, experts from multiple disciplines contributed to the assessment through the Net-Zero-2050 cluster of the Helmholtz Climate Initiative. This includes experts with knowledge of bioenergy with carbon capture (BECC), direct air carbon capture (DACC), enhanced rock weathering (ERW), geological carbon storage (S), and enhancing natural carbon sinks. Based on information from the literature and expert elicitation, the assessment was conducted in an iterative process using the indicators and traffic light system defined by the assessment framework (Förster et al., 2022). In total, the assessment and review process involved 24 experts with a background relevant for the CDR options including natural sciences (in particular related to physics, environment and climate), social science (in particular related to economics, policy and law) and interdisciplinary expertise in engineering, business management and sustainability. Where necessary, external experts were involved in the assessment (see Supporting Information S1 for further information). The CDR options used by Mengis et al. (2022) and described by Borchers et al. (2022) were jointly assessed by two groups of experts. The first group consisted of scientists with expertise in the respective disciplines of the dimension related to the feasibility of CDR options. The second group consisted of scientists with expertise in the development and application of the respective CDR option. In an iterative process, the two groups assessed the feasibility of CDR options for each of the respective dimensions. Thereby, the first group of disciplinary experts facilitated the assessment process for their respective dimension in order to ensure the consistency of the assessment process across the CDR concepts. The second group of CDR experts reviewed the ranking of each indicator according to the traffic light system, building on knowledge and literature including the CDR options described in Borchers et al. (2022). The BECC and DACC options were assessed separately from the component of the geological carbon storage (S). The reason for this differentiation is that there are multiple options for BECC and DACC that are applied and tested, while options for geological carbon storage (S) are limited within Germany. The fully combined BECCS and direct air carbon capture and storage (DACCS) concepts have not been applied in Germany yet. This assessment approach ensured that the main components of CDR options were adequately addressed.
Following the scoping of CDR options from Borchers et al. (2022), we here give only a short overview of the general features of 14 selected CDR options for Germany, with detailed information and description of the options to be found in the aforementioned publication. First, we include two DACC and one ERW CDR options, which use chemical processes to capture CO2 out of the atmosphere. Furthermore, we include six bioenergy combined with carbon capture (BECC) options, which combine biological and chemical carbon capture and are therefore called hybrid options. To complete the BECC and DACC options, we added one concept for geological storage solutions for Germany, again based on Borchers et al. (2022). Finally, CDR options that capture CO2 through photosynthetic processes and accumulate carbon in above or below-ground biomass are described in the biological carbon capture section, which incorporates three concepts that involve changes in agricultural practices, and two concepts of ecosystem restoration (peatlands and seagrass meadows).
DACC and storage is a method of filtering CO2 from the ambient air in a two-step process: CO2 capture and regeneration (Heß et al., 2020). In our study, we evaluated two types of application of DACC systems: (a) in a rather novel, small scale use in existing heating, ventilation, and air conditioning (HVAC) systems (DACC-HVAC; Dittmeyer et al., 2019), and (b) in more conventional, industrial-scale DACC farms. Since DACC options are energy-intensive processes, the technologies are most effective if supplied with carbon-emission-free energy.
ERW captures CO2 through chemical reactions of atmospheric CO2 with carbonate and silicate minerals spread on agricultural soils in the form of powdered limestone or silicate rocks (Beerling et al., 2020). This CDR option is an acceleration of the weathering process of silicate rocks that occurs in nature on geologic time scales (Archer, 2005; Walker et al., 1981). Carbon sequestered in soils is expected to eventually leach out and be transported to the sea.
Bioenergy with CCS encompasses a wide range of technological options, all based on the same principle: First, CO2 is captured from the atmosphere by plants as they grow, then the biomass is converted by combustion, fermentation, biomass gasification or pyrolysis into energy or energy carriers, for example, electricity, heat, biofuels. The CO2 produced during these processes is chemically captured at the point source (i.e., the bioenergy plant) and can subsequently be stored in geological formations or long-life products. While BECCS is considered one of the most viable CDR options (Babin et al., 2021), there are still reservations regarding its potential impacts on land use and biodiversity (IPBES-IPCC, 2021), which is why the biomass source considered for BECCS options is of relevance. In the following, we will present six different applications of BECC, each to be combined with geological carbon storage.
Combustion of woody biomass for heat and power cogeneration (CHP) combined with carbon capture (BECC-WCom), repurposes previous coal-fired power plants to use woody biomass feedstock. The CO2 released as the exhaust is then chemically captured and can be concentrated and transported to geological storage sites. This option allows for repurposing existing infrastructure, continued central power and heat provision and the use of technologies, which has already been demonstrated in other countries (e.g., in United Kingdom the example of Drax Group (2018) might be appealing given the impending coal phase-out in Germany (KVBG, 2020)).
The same woody biomass could be used for slow pyrolysis for biocoal production (BECC-WPyr) at around 500°C (Tripathi et al., 2016). To increase the CDR potential of this option, the biocoal can be used in soil applications, where the carbon is stored for centuries (assuming production temperatures that support a high stability of the biocoal). The gas generated during the pyrolysis as a by-product (Tripathi et al., 2016) which can be chemically filtered for CO2 and further used for storage.
A third BECC option that uses woody biomass is gasification of biomass for biofuels production combined with carbon capture (BECC-WGas). In this concept, biomass is converted into syngas using dual fluidized bed technology. From synthesis gas liquid hydrocarbons are synthesized in the Fischer-Tropsch process. The by-produced heat is used to provide process heat and generate electrical power, covering the energy demand of the concept. The CO2 emitted during the production process is captured and made available for storage. The provision of biofuels provides the opportunity for fossil CO2 emission abatement, but here it is considered to be stored. The availability of sustainable lignocellulosic biomass limits the overall potential of wood-based BECC technologies, like woody biomass combustion, woody biomass pyrolysis, and woody biomass gasification, especially if importing biomass is not considered to be an option (Thrän & Schindler, 2021).
Another BECC option to consider is biogas production for the generation of heat and electricity combined with carbon capture. With the highest number of biogas plants in operation in Europe (∼9,000, FNR, 2020), it appears sensible to investigate this option as a potential technology for BECCS in Germany. In our study, we further distinguish three biogas-based options, each using different type of biomass: (a) A mixed biomass biogas plant based on 50% of waste and residues, 20% of cattle manure, and 30% of energy crops (BECC-MxBG; as described in Thrän, 2019). (b) The use of wet ecosystems like peatlands for paludiculture harvesting for biogas and bioenergy production combined with carbon capture (PalBG) (Wichtmann et al., 2015). (c) Macroalgae farming for bioenergy production with carbon capture (BECC-MABG) that uses “offshore rings” located in the German North Sea exclusive economic zone (Buck & Buchholz, 2004; Fernand et al., 2017) for cultivation of brown macroalgae. The biomass would be harvested once a year and transported to biogas plants close to the coast. For the latter two biogas-based BECC options, limitations are related to location, as BECCS in combination with macroalgae and paludiculture can preferentially be used in areas that provide respective biomass, that is, marine areas or rural areas with specific biophysical conditions.
According to the Federal Institute for Geosciences and Natural Resources (BGR), deep saline aquifers and depleted gas fields are regarded as Germany's most relevant offshore and onshore solutions for storage.
Given the study's boundary conditions, we considered onshore CO2 storage. To ensure permanent storage, CO2 must be kept at depths >800 m in a supercritical state (IPCC, 2005). The injected CO2 remains trapped in the reservoir through various mechanisms, which vary depending on the specific storage location, and support long-term secure and effective CO2 storage (Kempka et al., 2014). Germany's Carbon Dioxide Storage Act (KSpG, 2012) currently prohibits underground CO2 storage. However, the law has recently been evaluated, and lifting the existing limitations is being considered (Bundesregierung, 2022). An alternative for permanent CO2 storage in Germany is transporting CO2 abroad to large-scale offshore projects in the North Sea (e.g., in Norway, Denmark or the Netherlands).
Practices that either restore or manage ecosystems aim to increase biological CO2 capture and sequestration. Changing agricultural practices has a large potential to increase soil carbon sequestration. An example is the afforestation of croplands (agricAFF). This conversion increases the annual carbon sequestration of unproductive lands that currently hold winter crops. Soil carbon accrual can also be enhanced by improving crop rotations (agricCR) to crops with a higher humus balance (Kolbe, 2012). This involves increasing crop residues and favoring crop varieties with deep and dense root systems (Don et al., 2018; Kell, 2011). Finally, including cover crops (agricCC) in the cropping cycle can increase soil carbon (Poeplau & Don, 2015). In Germany, about 2.2 million ha of arable land are already cultivated with cover crops (DESTATIS, 2018; Griffiths et al., 2019). A further 2 million ha of arable land (for potatoes, sugar beet, summer cereals, and maize) could be suitable for intercropping.
Peatlands are wetland areas in which water-saturated conditions facilitate natural accumulation of thick layers of decayed organic matter (peat) (Joosten & Clarke, 2002; Rydin & Jeglum, 2013). More than 98% of organic soils in Germany (approximately 1.8 Mha) are drained mostly for agricultural use. That results in 43 Mt of CO2 emissions each year (Tanneberger et al., 2021; Trepel et al., 2017). Hence recent efforts for peatland restoration were increased, since rewetting peatlands (PReW) offers the potential to increase carbon sequestration with additional benefits to the ecosystems.
Seagrass meadows are already mitigating emissions by absorbing CO2 through photosynthesis and by trapping particulate organic matter from the water, which gets buried in the sediment. They occur on the tidal flats of the southeastern North Sea (mostly the dwarf seagrass Zostera noltii) and the German Baltic coast (sublittoral seagrasses, here Zostera marina). An expansion of seagrass meadows, induced by human intervention (like planting or seeding) (SeaGr) to enhance the seagrass area can contribute to enhanced carbon burial (Lange et al., 2022) with benefits to marine biodiversity.
The assessment of the CDR options for Germany follows the suggested framework by Förster et al. (2022) along six dimensions. In the following, we will give a short overview of the indicators considered in the environmental, technological, institutional, economic, societal and system utility dimensions (for an overview of the assessment framework and the respective evaluation scale, see Förster et al., 2022).
The environmental dimension assesses how the deployment of a CDR option could potentially affect the atmosphere and terrestrial, aquatic and marine ecosystems. The impact variables are in line with commonly used impact assessment metrics (UBA, 2020). Effects on the atmosphere include emissions from changes in terrestrial and marine ecosystems, local climatic effects and noise. Effects of CRD deployment on terrestrial, aquatic and marine ecosystems are assessed in terms of spatial demands and related trade-offs, effects on biodiversity and soils as well as effects on water quality and quantity.
The technological dimension assesses the potential for deployment and upscaling of CDR options based on technological performance. This includes the efficiency of a CDR option in particular in terms of energy use (net energy balance) and capacity for CO2 removal (CO2 reduction and removal efficiency per energy unit). Market maturity is determined by the TRL as well as the compatibility with existing infrastructure. Lastly, the compatibility with the future energy system is evaluated with respect to the CO2 collecting effort and the ability to access low carbon energy carriers.
The economic dimension relates to costs of deploying CDR options, the effects this has on the domestic economy and possible barriers for CDR investments. Accordingly, the marginal cost for removing CO2 from the atmosphere is included in the assessment of the market costs, that is, the business cost of a given CDR option at this point in time. As costs of a CDR option can change over time, this is likely to alter also their relative cost vis-à-vis other CDR options, which is considered by also assessing the dynamic cost efficiency. This is done by including future cost reductions due to technological enhancements, cost reductions per unit of CDR when upscaling the production (economies of scale), and the marketability of co-produced goods (indicating economies of scope). External effects of CDR options, that is, impacts on third-party actors that are not taken into account by the actor causing them (e.g., negative or positive impact on water quality) are also considered in the economic dimension but are assessed in the environmental dimension to avoid double consideration in the assessment. Another cost category analyzed is transaction costs related to CDR deployment (e.g., for market screening, access and transaction, insurance and meeting regulatory requirements). The assessment includes transaction costs occurring for regulators and for actors involved in deploying CDR measures. The effects on the domestic/regional economy are assessed in terms of additional domestic value and employment. Investment barriers to CDR options are assessed by the share of capital cost in total cost (capital intensity), the specificity of the investments, and the revenue risk.
The institutional dimension addresses the policy landscape in which CDR options have to operate, taking a political and legal perspective on the maturity of CDR options and the feasibility of deploying CDR within existing laws and regulations, administrative capacities and accounting frameworks. Political (and institutional) maturity assesses the CDR options' position in the policy cycle (e.g., agenda setting, adoption of legislation, policy evaluation). The political acceptability is assessed by public and policy support for CDR options within the political debate, governmental support for research of a specific CDR option, as well as by the level of recognition of the role of CDR climate strategies at national and regional scale. Legal and regulatory feasibility addresses possible legal conflicts related to CDR options. It may be assessed by potential conflicts with existing legal requirements, the CDR options' conformity with human rights, and various environmental and conservation laws, particularly with climate laws. The assessment also addresses the demand for additional regulatory effort. Finally, transparency and institutional capacity include the assessment of existing monitoring, reporting, and verification (MRV) systems, the integration of CDR in national reporting of carbon emissions, and the integration of CDR in carbon markets. Beyond that, the institutional capacity is also assessed by the presence of capabilities for using adaptive and responsive approaches for governing the deployment of CDR technologies and whether the deployment of a CDR option requires additional administrative effort.
The social dimension assesses how CDR options are perceived by the public, the social context, associated costs or benefits in societal terms, the extent to which stakeholders are included and can participate in CDR deployment, as well as ethical implications. The public perception of CDR options evaluates the perceived risk of a CDR option, and the trust in institutions, as this has been shown to be a cause for resistance to technology deployment (Markusson et al., 2020; Waller et al., 2020; Winickoff & Mondou, 2017). The assessment of social co-benefits or costs includes potential impacts on health and employment. Inclusiveness and participation are found to increase public trust in technological projects and are assessed by the participation of the public during the planning and execution steps, the dialog on national and regional levels, and the transparency throughout the process. Ethical considerations are assessed by evaluation of the discursive legitimation, the CDR options' effect on intergenerational equity/justice, as well as regarding ethical reservations of resource use. The social context of CDR implementation is assessed by previous experiences with large-scale development projects and the corresponding local narrative.
The system utility dimension describes the potential of CDR options to remove emissions necessary to close the gap for achieving a net-zero CO2 system in 2050. Taking factors like the availability of biomass and the number of bioenergy plants attainable for retrofitting (relevant for BECC), costs and access to renewable energy supply (relevant for DACC), and available area (relevant for biological options) into account, we attempted to estimate the CDR potential within the German context. CO2 emissions avoidance potential is assessed by the amount of avoided current emissions to the system in the short and long term, respectively. Emissions potentially avoided in the future are not considered. For assessing the permanence of CO2 storage of a CDR option the natural persistence of the respective storage reservoir is considered in terms of decades, centuries to millennia (including risks due to natural and human-caused disturbances). CDR options are also assessed for the possibility to measure and verify their contribution to removing and storing CO2 as well as possible uncertainties involved in such estimates.
To present the results in an easy-to-read way, we introduce a traffic light system (see Förster et al., 2022) to indicate the effort required to overcome hurdles for the deployment of the assessed CDR options. Green indicates that the implementation of a CDR option is likely to be possible under current conditions (high feasibility) involving no or few hurdles for implementation. Yellow means that there are hurdles of medium magnitude to the implementation that require additional effort to be overcome. Red indicates that the implementation of a CDR option is currently not feasible (low feasibility) with considerable hurdles for implementation. In addition, we indicate if an indicator was “not applicable” for certain CDR options (gray), or if insufficient or ambiguous data was found for the assessment (white).
2.1 Selected CDR Options
2.1.1 Chemical CDR Options
2.1.2 Hybrid CDR Options—Bioenergy With Carbon Capture and Storage (BECCS)
2.1.3 Geological CO2 Storage Solutions
2.1.4 Biological CDR Options
2.2 Assessment Framework
2.3 Evaluation Scales
3 Assessment of the Individual Dimensions
3.1 System Utility Assessment
We find that relative to the removal need based on estimates of remaining emissions between 32 and 70 Mt CO2/year for Germany by mid-century (Kopernikus-Projekt Ariadne, 2021; Mengis et al., 2022; UBA, 2021), seven out of 14 options are estimated to provide significant annual removal in the order of magnitude of 10% or more of remaining emissions (F1 is yellow or green, Figure 1). More specifically, our estimates for BECC-based CDR potentials range from 0.5 to 29.9 Mt CO2/year, where paludiculture and macroalgae for biogas CHP (0.5 and 0.8 Mt CO2/year, respectively) show the lowest removal potential, and mixed biomass for biogas CHP, wood biomass for pyrolysis for biochar production and woody biomass for combustion CHP (12.6, 14, 29.9 Mt CO2/year, respectively) show the highest removal potential (Borchers et al., 2022; see Supporting Information S1 for details). If we assume that DACC in heat, ventilation and air-conditioning systems are installed in 15% of the largest buildings in Germany, the CO2 capturing potential would amount to 15 Mt CO2/year. If constrained by renewable energy supply by mid-century DACC-farms carbon removal potential would be limited to about 16 Mt CO2/year (Kopernikus-Projekt Ariadne, 2021). All BECC and DACC options would have to be combined with geological storage for which the storage capacity in discontinued oil and gas fields amounts to an order of magnitude of 2.200 Mt CO2 (Michael et al., 2011). In addition, saline aquifers on and off-shore could hold another 20,000 Mt CO2 (Knopf & May 2017). Finally, the scaled potential of natural sink enhancement (NSE) CDR options in Germany was estimated to range from 0.1 to 6.3 Mt CO2/year, where seagrass restoration and cover crops on agricultural soils show the lowest removal potential (0.1 and 1.7 Mt CO2/year, respectively), and terrestrial enhanced weathering, and improved crop rotation on arable soils show the highest removal potential (4 and 6.3 Mt CO2/year, respectively; Borchers et al., 2022; see Supporting Information S1 for details).
Some of these CDR options bring about the additional systemic effect of emissions avoidance (F2, Figure 1). This is true for almost all biomass- and biogas-based bioenergy CHP options, where fossil coal or gas can be replaced by biogenic fuels thereby reducing emissions for electricity and heat production (Borchers et al., 2022). For the rewetting of peatlands the systemic effect of emissions avoidance could be up to 43 Mt CO2/year by 2050 (Tanneberger et al., 2021), which is found to be more relevant than the removal potential. Noteworthy is the opposite effect of emissions avoidance for the chemical carbon capture options, for which their high energy demand especially in the near term would likely cause an increase in fossil emissions (F2 is red, Figure 1).
Concerning the durability of carbon storage and risks by anthropogenic or natural perturbations (F3, Figure 1), the DACC and BECC options rely on geological storage, for which several thousands of years of storage with close to zero leakage and low natural risk of perturbations are found (Banks et al., 2021; Kempka et al., 2014). Noteworthy is the higher risk of anthropogenic recovery of the stored CO2 for later usage, if depleted oil and gas fields were to be used for CO2 storage. Both pyrolysis and gasification of biomass produce products, for which we assume storage, but which bear a risk of anthropogenic usage. For the CDR options that do not depend on geological storage, durability ranges from thousands of years for enhanced weathering and rewetted organic soils (Löschke & Schröder, 2019; Borchers et al., 2022, respectively), over centuries to millennia for the seagrass meadows (Borchers et al., 2022), to decades to centuries for different agricultural practices to increase top soil carbon (Dynarski et al., 2020; Mutegi et al., 2013; Poeplau & Don, 2015). CDR removal based on natural ecosystems is more prone to carbon storage disturbances (e.g., Fuss et al., 2018; Poeplau et al., 2011). Climate change impacts and anthropogenic disturbances (e.g., changes in the occurrence of pest infestations, forest fires and land use change) may alter carbon permanence. For seagrass meadows, carbon storage is sensitive to storm events, ocean warming, and seawater depth and quality. Hence the degradation of seagrass could lead to large losses in its function of storing carbon.
All CDR options seem to be monitorable in principle (see F4, Figure 1). For CO2 storage in geological reservoirs, geophysical methods are widely employed to monitor possible leakages. For marine and terrestrial options increasing carbon stock, well-established measuring options for soil/sediment carbon stock changes exist. However, the uncertainty due to temporal and spatial variability within the carbon stocks reduced the overall accuracy with which CO2 sequestration and therefore gross negative emissions can be reported.
Evaluation matrix of systemic and environmental dimensions. Carbon dioxide removal options are described in the table “Abbreviations,” and the color code and ikons are given in the right corner.
3.2 Environmental Assessment
We find that for all biomass-based CDR options the indicator for area demand (A2.1) is key to determine environmental impacts: the higher the area demand for biomass production the more land use competition and environmental impacts are to be expected. This is in particular the case for the BECC option involving biomass combustion in power plants (WCom), which is expected to increase biomass demand and thereby area demand (A2.1 is red, Figure 1) to meet the combustion capacity. As a consequence, it is to be expected that WCom has negative environmental impacts in particular for biodiversity (A2.2; Birdsey et al., 2018; Schlesinger, 2018). In contrast, the BECC options of gasification of woody biomass to liquid fuel (WGas) and the pyrolysis of woody biomass for biochar production (WPyr) assume to be integrated in the current use of fuelwood without the need of increasing biomass production, likely causing no additional environmental impacts (A2.1 is yellow, Figure 1). The CDR concept of retrofitting available biogas plants with carbon capture technology (MxBG) includes the assumption that biomass use was to stay within current levels. However, competition for land and water (e.g., for irrigation) would persist and together with the use of fertilizers and pesticides, MxBG is expected to involve a range of negative environmental impacts (A2 and A3 are red, Figure 1). This concerns in particular negative impacts on water quality and biodiversity (e.g., Babin et al., 2021; Haakh, 2017; Kirschke et al., 2019; UBA, 2014).
CDR options involving changes in agricultural practices by introducing changing the land-use to forest (agricAFF), cover crops (agricCC) and adjusted crop rotation for enhancing soil carbon storage (agricCR) are expected to have a range of positive environmental effects by potentially enhancing biodiversity and water and soil quality (A2 and A3 mostly green, Figure 1; e.g., Thapa et al., 2018). In particular CDR options focusing on enhancing the carbon sink potential of ecosystems such as paludiculture for biogas and bioenergy production combined with carbon capture (BECC-PalBG), and the restoration of peatlands (PReW) or seagrass meadows (SeaG) are expected to have positive environmental impacts in particular for biodiversity, soil and water quality (A2.2, A3.1–A3.4 are green, Figure 1; e.g., Gaudig et al., 2014; Joosten et al., 2013; Reusch et al., 2021). This indicates that ecosystem-based CDR options are likely to create multiple benefits to the environment.
Synergies between CDR options could possibly be harnessed when combining CDR options involving ecosystem restoration with BECCS. Peatland restoration (PReW) combined with paludiculture for biogas and bioenergy production with carbon capture (BECC-PalBG) is an example, where ecosystems are restored and managed for enhancing soil carbon and biodiversity conservation, while at the same time also providing options for biomass production that can be used for BECCS. However, shortly after rewetting peatlands a peak in emissions of non-CO2 greenhouse gases like methane and nitrous oxide occurs (Tanneberger et al., 2021).
There are knowledge gaps and research needs in particular related to indirect environmental impacts related to indirect land use effects in the case of BECCS and indirect impacts from energy use in the case of DACCS.
In particular for biomass-based CDR options environmental impacts are site-specific and dependent on local conditions and the type of management practices applied. For this assessment, we assume that the applied CDR options would follow sustainable management practices that are in line with environmental regulations (e.g., not exceeding thresholds for the use of pesticides and fertilizers or avoiding leakage of chemical substances of technical appliances). However, already current land management practices come with significant environmental impacts and related negative impacts are therefore likely to continue to persist, as it is the case, for example, for the leakage of nitrogen to water bodies (Kirschke et al., 2019; UBA, 2014). As environmental conditions differ locally, the environmental impacts of CDR measures will have to be reassessed at site-level when moving from national feasibility studies to local scale implementation. The presented assessment using the traffic-light system indicates trends in environmental impacts that can be expected from CDR implementation. These will have to be complemented with site-based assessments in order to understand the location specific implications.
3.3 Technological Assessment
The energy requirement differs significantly between the CDR approaches (B1, Figure 2). Chemical CDR options are most energy consuming, as they must cover their energy demand by external supplies (e.g., Fasihi et al., 2019; Heß et al., 2020; Moosdorf et al., 2014). Although the carbon capture processes for both BECC and DACC are energy intensive, part of the heat and/or power production in bioenergy plants may be used on site to cover the demands of energy generation and CO2 capture processes, so that no additional energy input is needed. Furthermore, DACC comes with higher effort for CO2 capture than BECC, as almost its whole energy demand is related to the capture process, whereas in case of BECC only a part of produced energy is used for CO2 capture—from 15% to 33%, depending on the option: 15% for gasification (WGas), 20% for biogas options (**BG), 24% for biomass combustion (WCom), and 33% for pyrolysis (WPry) (e.g., Thrän et al., 2020). If combined with CO2 storage, the technology efficiency of BECCS and DACCS will further decrease, as there is energy demand associated with geological storage as well (e.g., Wiese & Nimtz, 2019). In comparison, biological CDR options have a very low energy demand, mainly needed for the initial implementation of the CDR option (e.g., Smith, 2016). Additionally, they do not have energy needs for capture and storage of carbon as those take place via natural processes (e.g., photosynthesis).
Biological CDR options also present the highest degree of maturity (B2 is green, Figure 2), as they are already deployed on different scales. Also, most of the BECC options are technically mature (B2 mostly green, Figure 2) and may build on already established bioenergy and infrastructure (Thrän et al., 2020). However, in case of macroalgae and paludiculture based BECC, the infrastructure for biomass supply would still need to be substantially developed (e.g., rewetting peatlands, launching offshore rings for macroalgae farming) (B3 is yellow/light red, Figure 2; e.g., Buck & Buchholz, 2004). Further development effort is also needed for DACC options to enhance their cumulative CO2 capture capacity (B2 is light green and light red, Figure 2). There are 19 DACC pilot plants in operation in other countries (e.g., in Iceland and the US; IEA, 2021), but only few small low-temperature-DACC modules (as necessary for DACC-HVAC) tested in laboratories, which makes this option ready for deployment within a decade or later (Dittmeyer et al., 2019; Heß et al., 2020). ERW have been tested in a few field studies, however, achieved mixed results indicate a need for further investigations (Andrews & Taylor, 2019; Löschke & Schröder, 2019).
Additionally, BECC and DACC need the integration of the carbon storage elements (see GEOSTOR, Figure 2), whether it be domestically or abroad. In Germany, many elements of storage infrastructure would still need to be developed, including determining the storage sites and construction of injection wells, preparation of the monitoring system around the storage location, and establishing CO2 collection networks to deliver CO2 to storage sites (B3, B4.1 are red, Figure 2).
Evaluation matrix of technological and economic dimensions. Carbon dioxide removal options are described in the table “Abbreviations,” and the color code and ikons are given in the right corner.
3.4 Economic Assessment
The business or market cost of CDR options can be a first indication of their value and is usually expressed as cost per unit of carbon removed (Fridahl et al., 2020). Marginal CO2 removal costs tend to be lower for biological options (C1.1 are mostly green in Figure 2), sometimes even negative costs are indicated, as in the case for cover crops (Fuss et al., 2018). Peatland rewetting is assumed to involve relatively low costs (Couwenberg & Michaelis, 2015), while afforestation of croplands shows a very wide range in cost estimates (Fuss et al., 2018). However, the marginal removal costs of biological options are highly side specific and thus cannot simply be transferred to the German context. Furthermore, ecosystem-based CDR options often require scarce land resources, with the exception of agricCC, which means that they tend to have high opportunity costs (see C1.2 mostly red, Figure 2). Similar considerations also translate to biomass-based hybrid options. In general, chemical and hybrid options are characterized by comparably higher marginal removal costs (Beerling et al., 2020; Heß et al., 2020; IEAGHG, 2013; Kearns et al., 2021; Strefler et al., 2018) as they rely on technological equipment and recurring costs for inputs (energy, feedstock etc.). Due to the hypothetical nature of some of the analyzed CDR options and/or incomplete, ambiguous or lacking information on their market costs in general, for the specific (technological) setting of the CDR options, or for the German context, it reveals to be difficult to give definite estimates on the marginal removal costs for a number of CDR options (C1.1 are mostly white for tech CDR options, Figure 2). However, the notion “no data” should not automatically be interpreted as there being no data at all on the cost of the respective CDR option (see details in Supporting Information S1).
In the evaluated CDR options, cost reduction potential by technological progress seems to be limited (C2.1 is red and yellow, Figure 2). In case of BECC higher potential is seen for CO2 capture, rather than the bioenergy generation, as the latter is delivered by mature technologies (e.g., combustion, pyrolysis). Moreover, part of the cost may also be covered by revenues coming from sales of jointly produced goods, for example, heat and electricity produced by BECC (C2.3 yellow for BECC, Figure 2). For DACC options, cost reductions of scaling up operations (economies of scale) are expected to be quite significant, since mass production of installations is likely to reduce its cost (Heß et al., 2020). In comparison, such aspects of technological progress and economies of scale are expected to have less potential for reducing costs in biological options.
Private transactions costs, for example, for using relevant markets, setting up necessary contracts and complying with regulations, tend to be moderate to high for most of the CDR options (see C3.2, Figure 2). For chemical and hybrid options transaction costs for the erection of plants as well as for establishing supply chains/markets for inputs and outputs play a major role. For biological options often the high number of actors involved drives the transaction costs if new regulations have to be complied with and new markets need to be used, which is partially caused by the scattered ownership of private forest and agricultural land in Germany. The same applies for example, to decentralized DACC in HVAC systems which includes a high number of actors when applied on a larger scale as well as a larger number of relevant regulations.
The potential for increases in domestic value added provided by the deployment of the CDR options seems rather limited. This is due to little value added potential in general (as e.g., in the case of cover crops or the management of (existing) seagrass meadows) or the fact that the manufacturing and/or installation of equipment is (partially) done by companies from abroad (which might apply e.g., for DACC and BECC options).
An important barrier to investments in the CDR options can be caused by the expectation of a high amount of sunk costs in case the investment fails. This risk increases with the capital intensity of the CDR option (i.e., the overall costs of the CDR measure involves a high share of capital cost), the specificity of the investment (i.e., the financial loss when assets would be applied for other purposes than the envisaged CDR option) as well as with the risks of the expected revenues. Due to low investment needs, biological options tend to possess a rather low capital intensity while hybrid and chemical options that require the erection of technical facilities come along with rather high capital intensity. However, as DACC appliances show high operating cost (due to their high energy consumption) their capital intensity tends to be lower compared to BECC options. Meanwhile, they show a very high specificity of investment, since the technical facilities can barely be used for other purposes and hence would be a stranded investment if DACC turns out to have no economic viability. The same applies to the equipment of existing bioenergy plants with carbon capturing facilities. Biomass-to-liquid plants could switch to the production of other gases for industrial use which makes their investment less specific than those of other BECC options. Since for biological options the carbon is often fixed in (marketable) biomass, selling off the biomass if the CDR case fails remains an option and reduces the specificity of the investment.
The assessment of the revenue risk is challenged by the fact that many of the CDR options do not generate CDR related revenues (as e.g., seagrass meadows) or are not established yet. Thus, the institutional setting of a potential revenue scheme is unclear by now (e.g., DACC or ERW). This puts a high revenue risk on such options from today's perspective. The revenue risk is lower for options that are remunerated for climate protection contributions by a fixed payment scheme such as the EU's common agricultural policy (which applies to afforestation of croplands (agricAFF) and cover crops (agricCC)). BECC options are assessed to have a moderate revenue risk, as technology-related risks are rather low due to the high maturity of these technologies. However, BECC revenues partially are dependent on the development of the EU emissions trading system, which has shown a high volatility in the past and is subject to political discretion, thereby putting a certain risk on the revenues of these facilities. In the case of macroalgae as a feedstock the revenue risk can be assumed to be higher since failing algae yields in Germany (e.g., due to pests or technical challenges) can barely be substituted as established markets are missing.
3.5 Institutional Assessment
In general, institutional arrangements, policies, and laws are more developed for established measures considered as CDR options. For example, land use practices involving paludiculture for biogas and bioenergy production combined with carbon capture (BECC-PalBG), afforestation (agricAFF), enhancing soil carbon sequestration through peatland rewetting (PReW) and cover crops (agricCC) are already practiced and implemented today. These options are also characterized by greater acceptance in the policy debate (E2.1), conformity with existing regulations concerning human rights (E3.2), environmental laws (E3.3) and climate laws (E3.4). Hence, the regulatory effort related to these CDR options is comparatively low (E3.5) (see Figure 3).
Evaluation matrix for institutional and social dimensions. Carbon dioxide removal options are described in the table “Abbreviations,” and the color code and ikons are given in the right corner.
However, this is not the case for CDR options involving carbon capture and storage (CCS). BECCS and DACS options consist of multiple components with BECCS including land use for biomass production, bioenergy generation and DACCS requiring technologies for air capture and ultimately technologies for CCS. Different institutional arrangements apply for each of these components. Accordingly, these more complex CDR options require a diversity of institutional arrangements that can pose hurdles to CDR implementation.
In the case of BECCS, the components of bioenergy generation are already well established. Hence the current policy landscape and institutional arrangements facilitate the implementation of the bioenergy component of BECCS. However, this is not the case for the carbon storage (S) component. For example, the federal states of Mecklenburg-Vorpommern, Lower Saxony and Schleswig-Holstein have completely excluded carbon dioxide storage for their territories (Deutscher Bundestag, 2018). The reason is that carbon storage is highly contested in the public and policy debate in Germany (E2.1), with policies and institutional arrangements currently not supporting the implementation of carbon storage. Hence, the geological storage of carbon (GEOSTOR, Figure 3) is rather in an early stage of the policy cycle (E1.1). This is also true for DACCS: while the technologies for DAC are being tested, the CCS component is restricted by the lack of implementation options for carbon storage. Accordingly, the CCS component of BECCS and DACCS is currently limiting the application of these CDR options in Germany. This is reflected in the German National Climate Strategy, which indicates that the potential for CCS options should be examined but it does, however, not explicitly call for the implementation of BECCS and DACCS options (BMUB, 2016) (E2.3). Nevertheless, all CDR options are currently assessed through government-supported research (E2.2).
The same applies to the Monitoring Reporting and Verification (MRV) systems for CDR options (E4.1). While components of MRV systems exist for land-use related CDR options (paludiculture-based biogas CHP—PalBG, afforestation of croplands—agricAFF, peatland rewetting—PReW), there is no MRV system for BECCS and DACCS options. Hence these options are also not integrated into the carbon market (E4.3).
Knowledge gaps exist in particular with a view to those CDR approaches which are in an early stage of development such as ERW or seagrass restoration (SeaG) (Figure 3). Empirical research on other technologies whose results can be used for extrapolation is largely missing. In addition, the institutional aspects are difficult to quantify and the assessment remains tentative.
3.6 Social Assessment
Assessment of the social criteria is challenging, as societal dimensions affected by the different CDR options are subject to diverging definitions and inherent heterogeneity. The public perception of CDR approaches for instance results from different perspectives of stakeholders as that can be classified as individuals, households, industries and economic sectors, or the government. Individual perspectives are shaped by different preferences and circumstances and are furthermore dynamic and can change out of intrinsic or external motivators. In most cases, policy shapes the framework in which the different CDR concepts are presented, but diverging preferences about or exposure to concepts, knowledge or availability (from a technological or economic side) influences perception, acceptance, participation, and contexts the options can be assessed in.
As a result, the assessment is often lacking data or providing ambiguous information about CDR options. This applies especially to the social context (D5), where, due to the different TRLs, assessment of previous experience or local narratives is not available, although it is stated that for example, acceptance of technology options increases if there is exposure and past experience (Wüstenhagen et al., 2007). Acceptance, which can be understood as a consequence of successfully considering the social dimension (Figure 3), is crucial for successful implementation of options. For inclusiveness/participation, data is sparse and ambiguous for for example, paludiculture-based biogas CHP (PalBG), where national dialogues exist. Still, transparency is high only for the biomass part, but low for carbon capture, which leads to the category classified as medium (D3.3 yellow). Also, participation is, as it is a key measure to foster acceptance (Stadelmann-Steffen & Dermont, 2021), difficult to assess due to data availability and implementation status.
As for the hybrid and chemical solutions co-benefits can be found for gasification and paludiculture-based options regarding health and economic co-benefits for employment through increased business opportunities. This is also the case for macroalgae-based biogas CHP (MABG), ERW, and geological carbon storage (GEOSTOR). Employment co-benefits can also help in lowering societal barriers to acceptance, but ambiguous or economically detrimental effects from losing jobs, often indicating a structural change, can societally affect options negatively. Perceived risk for hybrid options and for storage options is also rather high, which is partly mirrored in issues with ethical considerations. This applies especially for geological storage, where social reservations are high, possibly due to no exposure and lacking knowledge and transparency. Looking at BECC options, there exist considerable barriers, as uncertainty regarding the effects, which are often paired with significant negative actions (e.g., competition for land use among options and natural resources in general), harm acceptance. Ethical resource use is the major issue here, as treating hybrid CDR options as a mitigation deterrence shifts the mitigation burden away from other sectors (Carton et al., 2020). For DACC, the resource use can compromise energy security, which is also an ethical concern that as a last consequence, affects acceptance negatively.
Regarding tendencies of the assessment of the options, the social dimension of biological options involving NSE is overall more positive than for hybrid or chemical options, where no clear-cut picture can be made. Health as a co-benefit of the options, meaning additional recreational use or better air or water quality often goes hand in hand with options also posing lower perceived risk. This applies for example, to afforestation (agricAFF) or restoration of seagrass meadows (SeaG). CDR options like these are also rated better considering ethical matters of intergenerational equity (D4.2) or through discursive legitimation (D4.1). This is something that applies to most nature-based solutions, as they are societally less invasive, so acceptance is granted easier. Among the hybrid options, paludiculture- and macroalgae-based biogas CHP (PalBG and MABG) are the ones with the overall most positive outlook, as co-benefits and inclusiveness increase the feasibility of the social dimension. However, such options for more ecosystem-based solutions also require land, which can lead to land use conflicts and lower acceptance by certain land user groups. Tampering with nature is socially frowned upon, which can be an additional reason for barriers in acceptance (Wolske et al., 2019).
4 Cross-Dimensional Assessment of CDR Options for Germany—Insights Into Hurdles, Opportunities, and Research Needs
The extent to which emissions are reduced and avoided in the coming years and decades strongly determines the amount of annual CO2 removal that is necessary to reach net-zero CO2 by mid-century (Mengis et al., 2022; Merfort et al., 2023; UBA, 2020). And while the implementation of CDR options is already part of the national climate strategy in Germany (KSG, 2021), currently CDR options considered in Germany's climate protection law remain limited. This is undoubtedly related to considerable knowledge gaps on the implications of CDR implementation and upscaling (BMUB, 2016). In an attempt to fill some of the knowledge gaps, we present here a holistic assessment of 14 CDR options in Germany, pointing to possible opportunities (green in the evaluation matrix), hurdles (red) as well as research needs (blank) (see Figure 4). Selecting relevant CDR options for Germany, we aimed to provide insights into their possible implementation, yet acknowledging that the local (sub-national) contexts of implementation can differ greatly (Rhoden et al., 2021).
Overview of the assessment. The assessment indicators of each dimension and carbon dioxide removal option were sorted according to their feasibility assessments from high implementation hurdles (red), over medium (yellow) to low or no implementation hurdle (green).
For BECCS options, we found that the CDR potential within Germany is significant, reaching up to 60% of Germany's residual emissions if combined (assuming residual emissions of 60 Mt CO2/yr, Mengis et al., 2022). Furthermore, owing to the heat and energy provision these concepts would allow for further emissions avoidance by displacing fossil emissions. Most bioenergy concepts have a comparably high TRL, with the exception of marine- and paludiculture-biomass feedstock options, which require further on-site development and testing. Concerning the infrastructure compatibility, we found low hurdles for implementation, especially for the biogas concepts as the existing infrastructure in Germany could be retrofitted with CO2 capture units, lowering the initial investment costs. However, the upscaling of related technology and infrastructure will require time and resources.
Environmental impacts of BECCS options are mainly related to resource demand. Where the demand for land, the type and intensity of land use involved, and the quantity of biomass or energy the upscaling of the CDR technology requires, would determine such impacts. Small-scale solutions within the current regime of biomass use from forests, would likely not increase environmental impacts of current biomass use. However, biomass production involving intensive agricultural land uses (e.g., growing bioenergy crops) for bioenergy generation, would have detrimental environmental effects from the use of fertilizers and pesticides. In particular, biodiversity, soil and water quality are impacted, which means external costs might be associated with these options. What is more, an increase in biomass demand poses the risk of causing indirect land use change effects within and outside Germany, as it would increase area demand for biomass production that might displace other land uses like food production or nature conservation. This would negatively impact the enjoyment of certain rights such as the right to food and water, as well as the right to property (Mayer, 2019).
A major caveat of the assessment is the inability to account for resource competition between the different CDR options. While some of the options could be implemented simultaneously without having obvious mutual interference, others might compete for the same resources. This is true for some of the BECC concepts that rely on wood as a feedstock, and it especially applies to the competition for land—a resource that is extremely scarce in densely populated Germany. Such resource competition not only means that not all of the CDR options might be applicable to their entire theoretical potential but also that there may accrue price effects from resource competition by the different CDR options that are not considered when estimating future costs of the CDR options separately.
For the DACCS options we identified a significant carbon removal potential in the order of magnitude of Germany's residual emissions. Its high scalability provides the possibility for economies of scale for DACC options. However, this potential is constrained by external factors, which in turn impact the feasibility within other dimensions. In contrast to bioenergy-based CDR options, technology readiness is lower for chemical CDR options, including ERW. While the technology for DACC and ERW exists and is being implemented in pilot sites, investments required for upscaling these technologies and the high energy demand are considerable hurdles. Energy supply plays an important role in particular for big DACC farms with typical size of approximately 1 Mt CO2/year. If deployed at large scale (tens to hundreds of farms), associated energy demand, preferably coming from low-carbon sources, could possibly outnumber supply. For DACC, the direct environmental impacts from the technical installations are considered low as their spatial demand is low. However, the main environmental impact from DACC will be determined again by their high energy demand and the type of energy source used. Environmental impacts are expected from the additional energy needs that come with impacts on air and water quality and water demand.
Most crucially, BECCS and DACCS options would need to be combined with new CO2 transport and storage infrastructure to provide negative emissions. Now, within the German context, geological storage is a highly contested topic among the public and within climate policy debates. Engaging the public in a debate on CDR and using approaches for the co-creation of respective projects may generate more acceptance. In addition, laws are currently restricting underground CO2 storage at pilot-scale sites with no new storage sites being proposed at the moment (KSpG, 2012). Geological CO2 storage might be less contested by the public if considered outside of Germany. Currently, the lack of public acceptance as well as regulation prohibiting the implementation of geological storage within German territory, pose a substantial hurdle for BECCS and DACCS implementation. Furthermore, if these hurdles were to be overcome, the need for expanding CO2 transport and storage infrastructure is likely to cause additional delays in deployment. This also poses a risk for sunk cost due to the specific nature of the investment which might translate into investment restraint. Such delays negatively impact the short-term deployment of the CDR options with most “high-tech” options likely to require five to 10 years for achieving market readiness. Given the expected cumulative contributions by BECCS and DACCS to CDR until 2050, any delay in implementation is increasing their expected contribution over time. Furthermore, we identified a high risk of anthropogenic disturbance related to carbon capture methods involving products like bio-coal, biofuels, or synthetic fuels with lower permanence as compared to geological storage for carbon removal. Environmental impacts of geological storage are partially uncertain, as they are strongly related to risks associated with underground storage, like leakage from wellbores or hydraulic fracturing of caprocks and contamination of drinking water due to pressure buildup in the storage reservoir (Kelemen et al., 2019). From a societal point of view, the possibility for large-scale CDR deployment like BECCS and DACCS options poses a risk for mitigation deterrence (e.g., Bellamy et al., 2021; Grant et al., 2021; McLaren, 2020).
For ecosystem-based CDR options in the German context, we find one option (improved crop rotation—agricCR) with the potential to cover 10% of the remaining emissions (assuming residual emissions of 60 Mt CO2/yr, Mengis et al., 2022), but most struggle to reach significant CDR potentials. This is not surprising given the area and hence upscaling limitations within Germany. Due to their area demand, competition over land-use and related opportunity costs can be a considerable hurdle. Again, a major challenge of the evaluation scheme is that the separate assessment of the CDR options cannot account for resource competition between the different CDR options. Furthermore, several ecosystem-based CDR options (afforestation of croplands—agricAFF, cover crops—agricCC and seagrass restoration—SeaG) were assessed to have a high risk related to climate change impacts as well as natural and human-caused disturbances, which enhance the uncertainties in the permanence of carbon storage in ecosystems.
Nevertheless, ecosystem-based CDR options (such as peatlands rewetting -PReW, changes in agricultural management of cover crops—agricCC, etc.) are already practiced, while others are awaiting routine use (seagrass restoration—SeaG). The analyzed ecosystem-based CDR options are already established, commercialized options (e.g., afforestation, agricultural practices, peatland rewetting) that can be upscaled within relatively short-term.
The market-readiness is likely linked to the fact that ecosystem-based CDR options have been seen as favorable compared to “high-tech” CDR options, as they are often perceived as less invasive or even beneficial in their nature. The environment assessment supports this, as ecosystem-based CDR options are found to have a low environmental impact and even improve some environmental indicators (e.g., biodiversity, soil and water quality) surrounding local areas of their implementation. However, competition for land can be a key constraint for ecosystem-based CDR options and ensuring that these options provide additional benefits is likely to be critical for their acceptance and economic viability.
This analysis provides a first comprehensive assessment of selected CDR options for Germany across multiple thematic areas and disciplines. However, the focus of the study comes with inherent limitations, which we would like to point to in this section.
First, given the rather coarse assessment scale of the traffic light system, this analysis often provides qualitative information on general trends related to the feasibility of CDR options within the German context. As the analysis is in part based on expert judgments, subjective views and biases cannot be excluded, and might deviate from other relevant stakeholder perspectives. Furthermore, as environmental conditions differ between sites, locally specific assessments could identify regional differences in the feasibility of CDR options. Therefore, site-specific assessments (e.g., as part of environmental impact assessments) are needed for better understanding the location specific implications. Locally more specific assessments of CDR options within a particular local context (e.g., pilot sites) might lead to different conclusions.
The comparability of the selected CDR options' assessment is limited due to the differences in the implementation scales with respect to their annual removal rate. While the maximum removal scale for each option was chosen, the fact that the annual rates vary substantially impacts among others the options environmental assessment for example, with respect to area demand and its associated impacts. Beyond that, a thorough assessment of the socio-political and legislative dimension would benefit from the development of context-specific implementation scenarios, including information on relevant actors, stakeholders and impacted communities.
Finally, the selected options are not a comprehensive list of possible CDR options for Germany, but was chosen based on the available CDR option portfolio from Borchers et al. (2022). In particular marine-based CDR options are under-represented in this exercise.
4.1 Limitations of the Study
5 Outlook—Lessons Learned
The direct environmental impacts of CDR options can be anticipated based on information already available for the different land management practices related to biomass production. However, for future assessments it is critical to address potential indirect environmental impacts across regional and global scales in particular when upscaling CDR measures.
In terms of technological maturity of analyzed CDR options, biological options represent the highest readiness for a near-term upscaling. Some of the BECC options are also technically ready but face legal constraints and lack of infrastructure for CO2 transportation and geological storage in Germany. DACC concepts additionally involve a high renewable energy demand, which is expected to be accessible only in the longer term.
With respect to the cost of CDR options, our analyses show that non-market costs like transaction costs and opportunity costs related to the implementation of CDR measures pose an important barrier to many of the CDR options. Their potential “invisibility” compared to market costs (e.g., for energy, labor, feedstocks and other inputs) bears the risk of being overlooked in the evaluation of CDR options. Therefore, (political) decision-makers should be aware of this potential evaluation bias and make sure that these non-market costs are carefully considered as well.
Public acceptance is a key aspect for successful implementation of CDR options. However, the assessment of social impacts of CDR options is difficult due to their heterogeneity, uncertainty, as well as largely missing data. The heterogeneity of the social dimension originates from the multiformity of the “public,” which includes different stakeholders with diverse preferences and experiences: citizens, industries, government. In politics, re-election matters, which is only possible, if concerns of the citizens are heard, which is also likely to influence decision-making on upscaling CDR options. Industry also has interest in favorable economic conditions, which might not align with the preferences of citizens. Hence politics plays an important role in shaping the framework for the implementation of CDR options.
Investigating support within the policy landscape, determining transparency and governance requirements and assessing the legal and regulatory feasibility of CDR options need to be addressed. For many CDR approaches this is more complex as they are at an early stage of development and there is uncertainty on how they will work in practice, at what scale they will operate and where they will get their energy from. Therefore, there remain important factors that could lead to conflicts with other policy goals. Potential future conflicts will hence depend on many other unforeseeable variables and will be difficult to predict. The law, however, usually responds reactively to social issues and conflicts that have gained a certain structure and clearly require legislative intervention. While guidance on future conflicts can at best be provided by extrapolating from similar cases and past experience, this could carry a potential for errors.
In total, about 5–15 Mt CO2/year could potentially be removed through ecosystem-based CDR measures, 15–20 Mt CO2/year by chemical capturing CDR options and 20–40 Mt CO2/year by BECCS CDR options by 2050 within the German context. Determining the short- and long-term CDR potential, as well as the avoided emissions potential of the CDR options, is a challenging part of their assessment, due to many assumptions related to their deployment. However, compared to the overall German CO2 emissions in 2020 of 644 Mt CO2, it becomes clear that the removal potential is still found to be relatively small and underlines the need for fast and effective emission reduction measures. While challenging, it is necessary to distinguish between removed and avoided emissions since the effects on the carbon accounting in the context of net-zero CO2 are very different. This distinction, together with separation of natural from anthropogenic sinks, allows for clearer communication of the net removal potential of CDR options and should be picked up by any national reporting system when implementing CDR.
Acknowledgments
The Helmholtz-Climate-Initiative (HI-CAM) is funded by the Helmholtz Associations Initiative and Networking Fund. The authors are responsible for the content of this publication. N.M. is funded under the Emmy Noether scheme by the German Research Foundation “FOOTPRINTS—From carbOn remOval To achieving the PaRIs agreemeNt's goal: Temperature Stabilisation” (ME 5746/1-1). NM thanks Christeena Babu for help with references and SI formating. MB, JF, DT are also grateful for funding provided by the BMBF Grant 01LS2107A (BioNet). We would like to thank anonymous external experts who assessed the social criteria based on their expertise by filling out a survey with queries about the social criteria and indicators. Open Access funding enabled and organized by Projekt DEAL.
Conflict of Interest
The authors declare no conflicts of interest relevant to this study.